Research
Our goal of research is to develop knowledge and innovative products that are impactful and beneficial for the society and humanwellbeing. The energy crisis, water scarcity and environmental deterioration are among the biggest challenges to be faced by the world in the 21st century. It has become critical for us to seek for better ways to fruitfully exploit the resources yet with minimized negative impacts on the environment. Motivated by this and our passion for thermal-fluid sciences, our research has primarily concerned novel studies of thermal-fluid problems from multiphase flows to porous materials and biomedical flows with applications in thermal management, low-carbon energy, health and climate change. We combine experimental, numerical, and analytical tools, such as microfluidics, particle image velocimetry (PIV), thermometry, and lattice Boltzmann method (LBM) to characterize transport processes in these flows. We also have extensive experience in microfluidics for studies of capillarity, biofilm, and additive manufacturing.
At M-LEFT, we strive to integrate a “small-to-large” approach to close the gap between basic research and real-world applications. Built upon thermal-fluid sciences and a unique tool set, we aspire to begin with fundamental physics at the nanoscale and work our way up to engineering systems. With that in mind, we have been growing our research efforts in three tiers: (i) fundamental research on micro/nano-scale energy and fluid transport; (ii) user-inspired research and technique development; (iii) engineering systems and technology transfer.
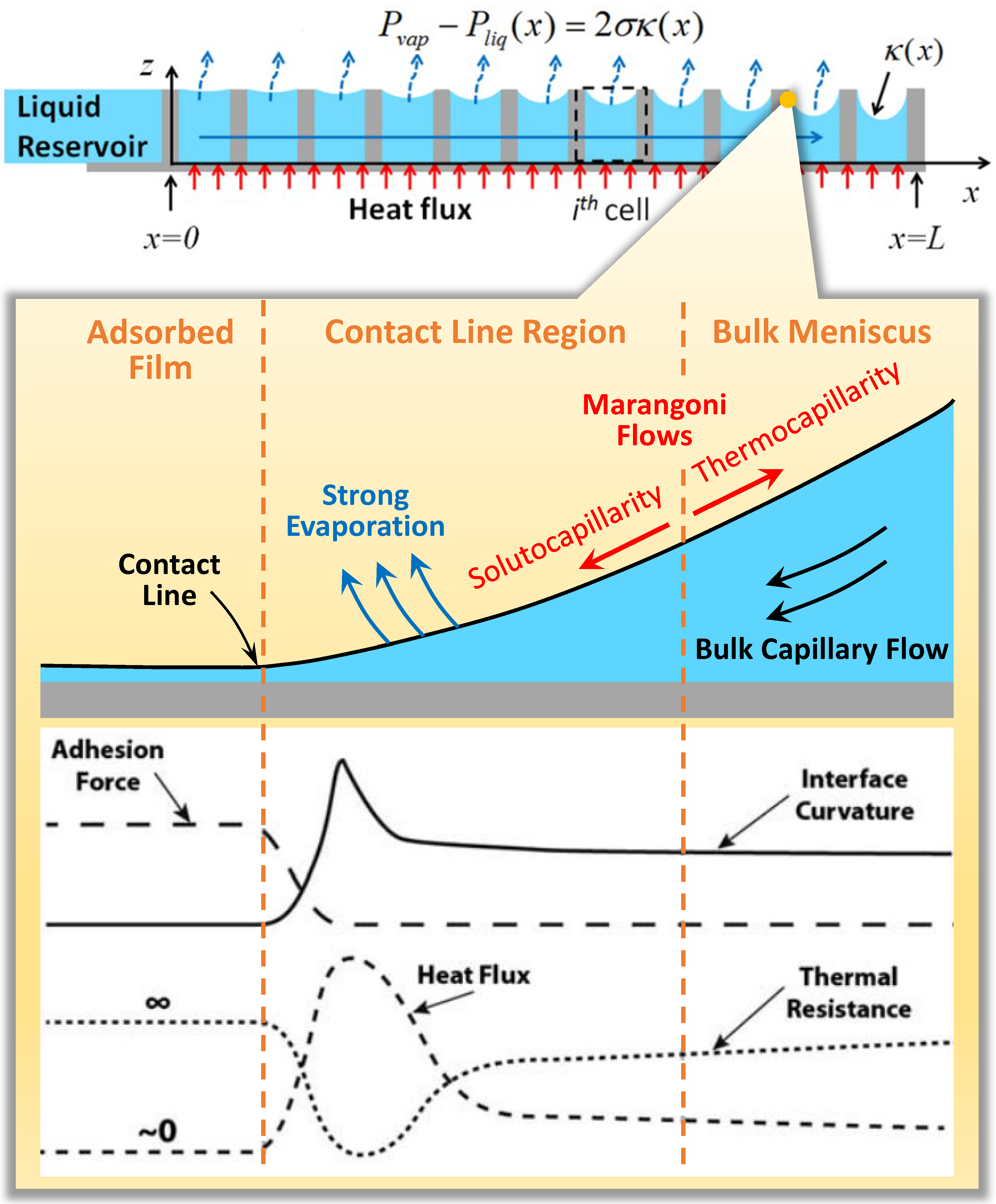
Thin film evaporation on micro-structured surfaces.
Electronics with ever-increasing power have become an indispensable part of our daily life. Effective thermal management is needed to cool these devices to a safe temperature while sustaining high performance with minimal adverse effects on the environment (Mahajan et al., 2006). Thin film evaporation, enabled by large latent heat, is a promising cooling strategy due to its simple design, high cooling capacity, and stability. By incorporating micro/nano-structures, the cooling capacity of thin film evaporation has been significantly improved (Hanks et al., 2018). However, it is still much lower than theory predictions, due to the lack of fundamental understanding of detailed flow and thermal characteristics at small scales. This project seeks to study fluid flow and thermal transport processes at evaporating interfaces by accurately measuring the flow speed, film shape and temperature within thin coolant films that are typically a few micrometers thick. Enabled by a set of innovative techniques with unprecedented resolution, this work will allow for accurate quantification of effects that are previously inaccessible such as temperature and velocity with nanoscale resolutions, thus filling in our current knowledge gaps. This five-year effort is sponsored by NSF. Related to this project, two NASA-sponsored projects focus on film flow characterization in pulsating heat pipes, and heat transfer mechanisms thermal protection system (TPS) materials. In the long term, we intend to expand this research direction to phase-change-based water and energy harvesting.
Below are some results showing the buoyancy-thermocapillary convection in a layer of a volatile silicone oil subjected to a horizontal temperature gradient. Under ambient conditions, where the volatile liquid layer is covered by a layer of air, the flow in the liquid layer destablizes in to single unicellular flow (SUF), partial multicellular flow (PMC), steady multicellular flow (SMC), and oscillatory multicelluar flow (OMC). The average concentration of noncondensables (air here) appears to have little effect on the base flow. The average concentration does, however, have a major effect on flow stability, and the critical Marangoni numbers for transition between the various flow states (SUF, PMC, SMC, OMC) increase as noncondensable concentration decreases, in surprisingly good agreement with the predictions of linear stability analysis.
Oscillatory multicelluar flow near the hot end.
Flow Regimes
Particle pathline visualizations of the flow in a thin layer of silicone oil subjected to various temperature gradients in the horizontal direction.
Related Publications:
- Li, Yaofa, and Mianmi Yoda. "An experimental study of buoyancy-Marangoni convection in confined and volatile binary fluids." International Journal of heat and mass Transfer 102 (2016): 369-380.
- Li, Yaofa, and Minami Yoda. "Convection driven by a horizontal temperature gradient in a confined aqueous surfactant solution: the effect of noncondensables." Experiments in fluids 55, no. 1 (2014): 1-11.
- Li, Yaofa, Roman Grigoriev, and Minami Yoda. "Experimental study of the effect of noncondensables on buoyancy-thermocapillary convection in a volatile low-viscosity silicone oil." Physics of Fluids 26, no. 12 (2014): 122112.
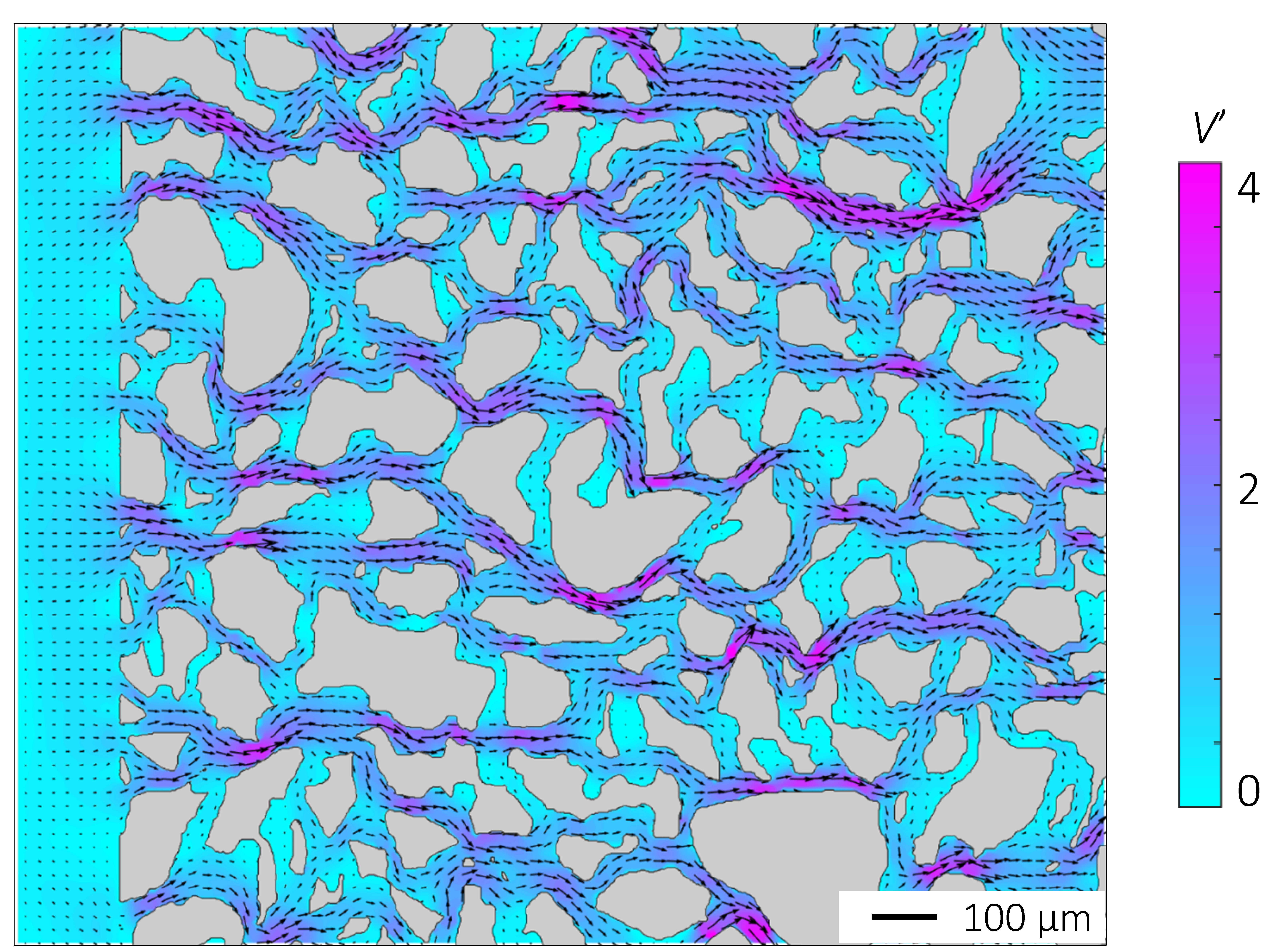
PIVIV measurement of water flow in porous media
Multiphase transport in porous media is central to a broad spectrum of water and energy-related processes. Typical applications include CO2 sequestration, enhanced oil recovery, and PEM fuel cells and electrolyzers. A better understanding of multiphase transport will help to strike the right balance between subsurface energy development and environmental sustainability. For instance, CO2 injections into hydrocarbon reservoirs have been successfully employed in enhanced oil recovery (EOR) activities for decades. Due to the very low viscosity of CO2, this technology is cost effective and appealing to large-scale operations. Recently, the scientific and technological interest in this topic has been renewed by the international effort to develop new solutions for reducing the global carbon footprint in order to contain greenhouse effects. Geological sequestration of CO2 into deep saline aquifers is considered a promising method to reduce CO2 emissions from large-scale fossil fuel power plants. In this regard, fundamental understanding of pore-scale physics in porous media flow is crucial to predicting the fidelity of candidate sites pre-injection of CO2 and its post-injection migration within the reservoir.
At the M-LEFT, we employ special techniques such as micro-PIV and epi-fluorescence microscopy to quantify the pore-scale dynamics and the phase distribution as a wetting phase (Water/Brine) is displaced by a non-wetting phase (CO2) in 2D porous micromodels under reservoir-relevant conditions. These noval experiments allow us to evaluate the importance of a few previously ignored phenomena, such as inertial effects and shear-induced motions. We showed that flows at pore scale really determine CO2 mobility at much larger length scales, and that instabilities driven by the competition between viscous and capillary effects may render inertial effects critical, which challenges traditional predictive models. We also team up with numericalists to quantify the interfacial dynamics, CO2 infiltration patterns and wettability effects using high speed fluorescent microscopy as well as powerful simulation tools such as Lattice-Boltzmann Method. Currently we have expanded our effort to the characterization of various other effects, such as capillary pressure, thin film flow, dissolution and precipitation.
CO2 displaceing water in a porous micromodel at high capillary number featuring viscous fingering
Related Publications:
- Rahman, Rafid Musabbir, Carson Kocmick, Colin Shaw, and Yaofa Li, “Quantifying multiphase flow of aqueous acid and gas CO2 in deforming porous media subject to dissolution”, arXiv:2301.00378 (2023).
- Li, Yaofa, Gianluca Blois, Farzan Kazemifar, Razin S. Molla, and Kenneth T. Christensen. "Pore-scale dynamics of liquid CO2–water displacement in 2D axisymmetric porous micromodels under strong drainage and weak imbibition conditions: high-speed μPIV measurements." Frontiers in Water 3 (2021): 710370.
- Li, Yaofa, Gianluca Blois, Farzan Kazemifar, and Kenneth T. Christensen. "A particle-based image segmentation method for phase separation and interface detection in PIV images of immiscible multiphase flow." Measurement Science and Technology 32, no. 9 (2021): 095208.
- Ilin, Eduard, Yaofa Li, Eugene V. Colla, Kenneth T. Christensen, Muhammad Sahimi, Maxim Marchevsky, Scott M. Frailey, and Alexey Bezryadin. "Nanoscale detection of metastable states in porous and granular media." Journal of Applied Physics 127, no. 2 (2020): 024901.
- Li, Yaofa, Gianluca Blois, Farzan Kazemifar, and Kenneth T. Christensen. "High‐speed quantification of pore‐scale multiphase flow of water and supercritical CO2 in 2‐D heterogeneous porous micromodels: flow regimes and interface dynamics." Water Resources Research 55, no. 5 (2019): 3758-3779.
- Fakhari, Abbas, Yaofa Li, Diogo Bolster, and Kenneth T. Christensen. "A phase-field lattice Boltzmann model for simulating multiphase flows in porous media: Application and comparison to experiments of CO2 sequestration at pore scale." Advances in water resources 114 (2018): 119-134.
- ChenYuYu, Yaofa Li, Albert J. Valocchi, and Kenneth T. Christensen. "Lattice Boltzmann simulations of liquid CO2 displacing water in a 2D heterogeneous micromodel at reservoir pressure conditions." Journal of contaminant hydrology 212 (2018): 14-27.
- Li, Yaofa, Farzan Kazemifar, Gianluca Blois, and Kenneth T. Christensen. "Micro‐PIV measurements of multiphase flow of water and liquid CO 2 in 2‐D heterogeneous porous micromodels." Water Resources Research 53, no. 7 (2017): 6178-6196.
Precise measurement and control of pressure with high resolutions in microfluidic systems are key to numerous scientific and engineering applications, ranging from sample manipulation in biological studies to the evaluation of capillary pressure in multi-phase flow in porous media (Chung et al., 2009). Our current ability for pressure mapping in microfluidic devices is limited, primarily due to the small sizes of typical microfluidic devices, where conventional pressure sensitive paints (PSP) cannot be effectively implemented. To this end, we are designing microscale pressure sensors by miniaturizing the conventional membrane-based design and incorporating innovative optical and electrical readout schemes.
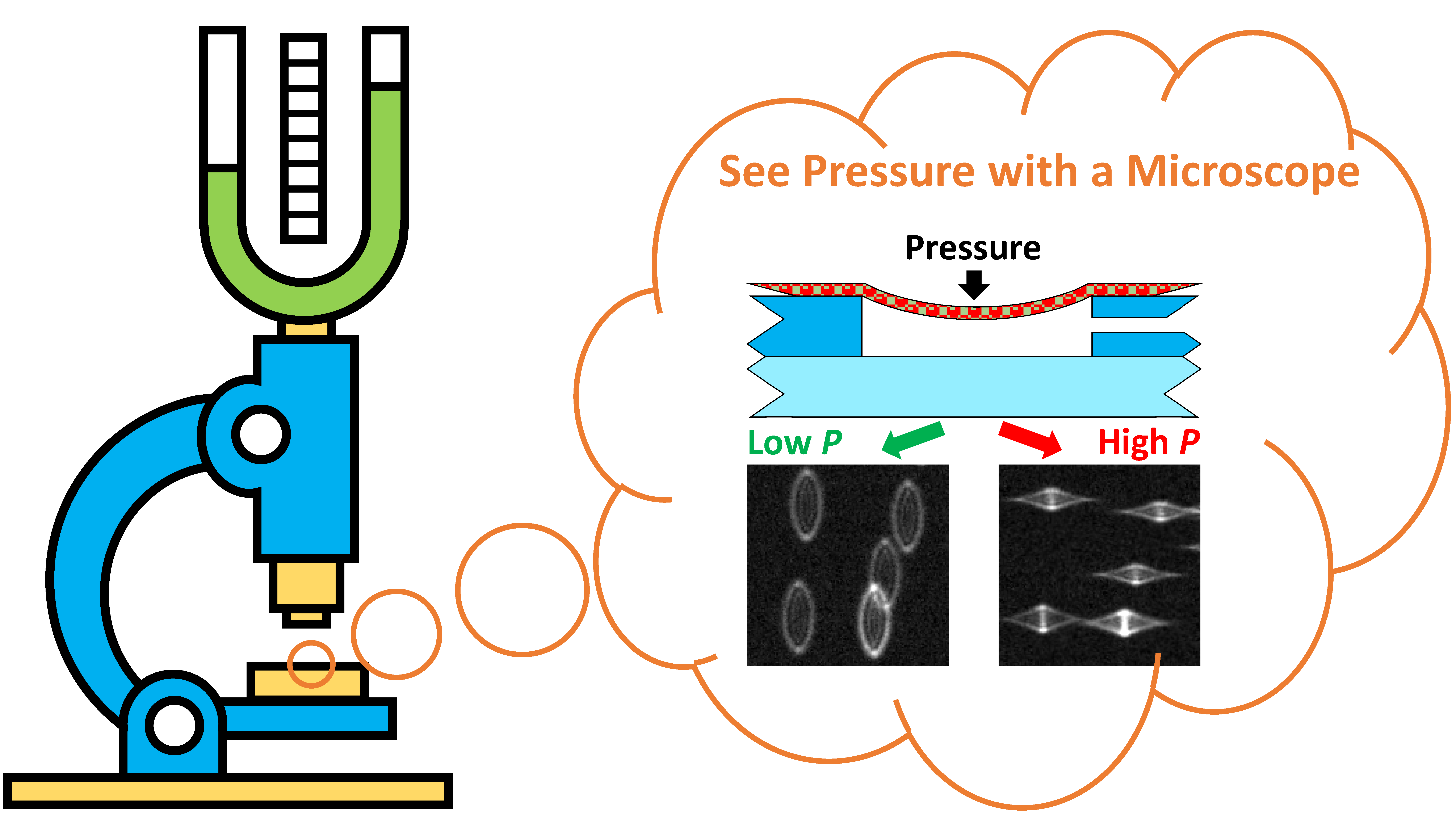
Pressure sensor principle.
Work featured on cover.
As a first step of this effort, we have successfully designed and fabricated a polymer-based sensor using simple soft lithography. By leveraging fluorescent particles and astigmatic particle tracking, the membrane deflection is detected optically based on the shape of the particles. The simple readout method and image processing algorithm have led to fast and precise pressure measurements under both single and multiphase flow conditions. This work is featured on the cover of Lab on a Chip (Raventhiran et al., 2022). The next step will be to further improve the accuracy and resolution by leveraging new materials such as silicon nitride as the sensing element. Our goal is to achieve an accuracy of 10 Pa and a spatial resolution of 10 mm. The sensor in development will provide a novel method for in-situ quantification of local pressure and thus opens the door to a renewed understanding of pore-scale physics and capillarity in multiphase flow.
Related Publications:
- Raventhiranan, Nishagar, Razin Sazzad Molla, Kshithij Nandishwara, Erick Johnson, and Yaofa Li. "Design and fabrication of a novel on-chip pressure sensor for microchannels." Lab on a Chip 22, no. 22 (2022): 4306-4316.